We've just about finished up all the technical details we can alone, and are beginning to work on outreach. As the blog is a truly horrible way to make details accessible, we're working on a MarMoSat website! It is very much a work in progress, but new information is being added all the time.
This means the blog will now function as a blog! Posts will be rare for the foreseeable future, but when they do come, they'll be broader and more stand-alone, talking about all sorts of Phobos-related stuff.
We are officially shifting out focus onto our orbiter-only concept, as it appears far more achievable. We are hoping that regardless of what happens with MarMoSat, we can use our experience with PALADIN to get involved with a secondary payload for JAXA's MMX mission to the Martian moons in 2024.
Thanks for staying with us!
-Daniel
A student-led project to design, develop, and build the first spacecraft to explore the Martian moons
Wednesday, June 7, 2017
Wednesday, April 19, 2017
Mobility update
A brief update on PALADIN's mobility system:
The above is an early animation of the deployment of PALADIN's wheels. Details are available at our earlier post on Mobility.
We are currently looking at motors for PALADIN. There are a number of options we're looking at, with the following criteria (including gearhead):
Runs on less than 1W (up to 1.5 W acceptable)
Output step size at most half a degree - preferably smaller
Output rotational speed at least 2 rpm - preferably higher
Mass under 25 g per motor - preferably ~10 g
Diameter under 30 mm - preferably ~10 mm
Length under 50 mm - preferably ~30 mmMinimizing step size allows careful positioning and keeps the rover in contact with the surface.
Maximizing rotational speed allows a higher maximum speed for longer traverses.
We are reaching out to space advocacy organizations and aerospace companies for support.
Phobos postcards! Thanks to Vistaprint. |
Coming up next, a lengthy post on why we want to explore the Martian moons!
-Daniel
Friday, April 7, 2017
Focus On: Power Subsystem
A brief note on MarMoSet's power:
Mars will be near aphelion, its furthest point from the Sun, when MarMoSet arrives. Being 1.6 times further from the Sun than the Earth is, solar panels would provide only 36% of the power they would in Low Earth Orbit.
Our propulsion system, the ConstantQ hybrid electrostatic thruster, requires about 22 watts to operate. The thrusters would have to fire for extended periods due to their low thrust, so solar arrays must provide at least this much power. For this reason, we plan to use MMA Design's eHaWK array, which is the largest off-the-shelf CubeSat solar array.
With two wings, each with three 20 x 30 cm panels, the eHaWK provides 26 watts of power even at Mars. The arrays are gimbaled, so they can track the Sun as MarMoSet orbits, ensuring consistent power.
Such large arrays pose challenges for PALADIN, the mission architecture that includes roving on Phobos's surface. Even if the arrays are strong enough not to be broken or mangled during landing, they could easily get caught on the terrain, prematurely ending PALADIN's mission. For this reason, the two large wings will detach from the spacecraft during its final approach of Phobos (confirmed feasible by MMA). Once landed, the nanorover no longer requires enough power to operate its thrusters, which in any case would not be capable of lifting it off Phobos's surface.
PALADIN (and possibly the orbiter-only architecture, DELPHI) would therefore also have a body-mounted solar panel on its upper 6U face. Depending on the model, this array would provide at least 4.3 - 7.2 watts at solar noon.
MarMoSet will also carry batteries to provide the spacecraft with power during nights on Phobos. We are still deciding how much storage is necessary, but are currently planning to store 40 Wh, enough to fire the thrusters at full power for nearly two hours and to provide PALADIN with power for a full Phobos day.
Depending on orbital planning and volume available, we may increase energy storage capacity. Two 10 Wh batteries would likely fit on either side of the NAC's optical tube..
That's all for now! We're currently working on flight computers, Mars capture, and nanorover motors, posts on each likely coming soon!
-Daniel
Mars will be near aphelion, its furthest point from the Sun, when MarMoSet arrives. Being 1.6 times further from the Sun than the Earth is, solar panels would provide only 36% of the power they would in Low Earth Orbit.
Our propulsion system, the ConstantQ hybrid electrostatic thruster, requires about 22 watts to operate. The thrusters would have to fire for extended periods due to their low thrust, so solar arrays must provide at least this much power. For this reason, we plan to use MMA Design's eHaWK array, which is the largest off-the-shelf CubeSat solar array.
![]() |
Credit: MMA Design |
With two wings, each with three 20 x 30 cm panels, the eHaWK provides 26 watts of power even at Mars. The arrays are gimbaled, so they can track the Sun as MarMoSet orbits, ensuring consistent power.
Such large arrays pose challenges for PALADIN, the mission architecture that includes roving on Phobos's surface. Even if the arrays are strong enough not to be broken or mangled during landing, they could easily get caught on the terrain, prematurely ending PALADIN's mission. For this reason, the two large wings will detach from the spacecraft during its final approach of Phobos (confirmed feasible by MMA). Once landed, the nanorover no longer requires enough power to operate its thrusters, which in any case would not be capable of lifting it off Phobos's surface.
PALADIN (and possibly the orbiter-only architecture, DELPHI) would therefore also have a body-mounted solar panel on its upper 6U face. Depending on the model, this array would provide at least 4.3 - 7.2 watts at solar noon.
MarMoSet will also carry batteries to provide the spacecraft with power during nights on Phobos. We are still deciding how much storage is necessary, but are currently planning to store 40 Wh, enough to fire the thrusters at full power for nearly two hours and to provide PALADIN with power for a full Phobos day.
![]() |
Credit: Clyde Space |
That's all for now! We're currently working on flight computers, Mars capture, and nanorover motors, posts on each likely coming soon!
-Daniel
Monday, March 20, 2017
Targets of Interest
Both moons are covered in a thick layer of dust. Due to their proximity, Mars, Deimos, and Phobos exchange this dust as ejecta when one body is impacted. The amount of dust exchanged is not known, but a careful investigation of Phobos's regolith may reveal information about Mars's history that cannot be found even at Mars. Phobos's trailing face receives large amounts of dust from Deimos. Due to the moons' small orbits, most ejecta thrown off a moon will return to it, although usually on the opposite side of the moon, spreading it rather evenly.
Very few surface features of Deimos are known, both because it is smoother due to a thicker layer of dust, and because fewer missions have taken pictures of it. The only remarkable feature is a large concavity in its south hemisphere. Only two craters, Voltaire and Swift, are named.
![]() |
Credit: NASA |
Phobos is pretty cool. Both moons have a layer of light dust at least a few meters thick, but Phobos's layer is thinner, so craters and other features are not "muted" as those on Deimos are. It is also closer to Mars, so it is in a more dynamic environment, taking more impacts from ejecta thrown off of Mars and undergoing larger tidal stresses.
Phobos's most obvious surface feature is Stickney Crater. Stickney Crater is massive, about nine kilometers in diameter. Keep in mind, Phobos itself is only two to three times wider! Learning more about Stickney is crucial to learning more about Phobos, as the Stickney impact event shaped Phobos as we see it today. The impact nearly shattered the moon, and sent it spinning for about 14,000 years. In fact, there is evidence that Phobos's original orientation was opposite its current orientation - the impact flipped Phobos! The impact also coated all of Phobos with a layer of ejecta over a meter thick. There is some debate over the age of Stickney crater, as different methods suggest drastically different ages, and more information is needed to determine its true age.
![]() |
Credit: NASA |
Stickney Crater is a promising site for future exploration, including crewed missions, as it would shield crews from radiation*.
Stickney's northwestern rim displays landslide features, which are an obvious dynamic process that can teach us about Phobos's development, and unearth fresher regolith that would ordinarily be buried. These landslides may also be hazards that need to be investigated before a crewed mission, as the severity and extend of landslides is unknown.
![]() |
Credit: NASA |
Both moons are very dark, about as reflective as asphalt. However, in the near infrared spectrum, colors can be discerned. The moons' dust layer is largely reddish, but a region within and east of Stickney crater known as the "bluish" unit is, significantly less red. This region is likely excavated regolith, and investigating the interaction between the color units may help clarify Phobos's interior composition; whether Phobos is primarily "blue", with a thin layer of "red" on the surface, or a mix of "blue" and "red" blocks.
Credit: Brown University |
![]() |
Credit: Journal of Geophysical Research |
![]() |
Credit: Brown University |
Phobos is strewn with large boulders called ejecta blocks, most of which are thought to have been thrown up by the Stickney impact. Investigating the weathering of these ejecta blocks can teach us about Phobos's history and environment. More importantly, while the dust that makes up Phobos's surface layer is definitely weathered by long-term exposure to solar radiation, and is at least partially comprised of ejecta from Mars or Deimos, ejecta blocks are likely representative of Phobos's interior.
The most prominent ejecta block is the Phobos Monolith, an 85 meter boulder. This monolith is our primary target due to its proximity to the Sub-Mars point. As any single solid ejecta block can be called a monolith, we unofficially call this the "Reynolds Monolith" after Alastair Reynolds, who, in addition to being a magnificent science fiction writer, had the Phobos Monolith play a critical role in his "Blue Remembered Earth" universe.
Other ejecta blocks are scattered across Phobos, and can be sampled opportunistically. One other notable block is what seems to be a pile of ejecta, which we unofficially call "The Cairn". While not composed of a single block, it appears to be considerably larger than the Reynolds Monolith.
![]() |
The Reynolds Monolith. Credit: NASA |
![]() |
The Cairn, a pile of ejecta blocks. Credit: NASA |
![]() |
An ejecta block on the Moon. Credit: NASA |
![]() |
Map of ejecta blocks. Credit: Journal of Geophysical Research |
Finally, Phobos is covered in unique grooves. There are many different theories as to the grooves' origins, including crater chains, paths of rolling boulders, and dust draining into fractures formed by outgassing, impacts, or tidal stresses. Any of these have strong implications for all of Phobos. Likely more than one cause is responsible for the grooves, as at least three different types of grooves exist, and most theories do not explain all grooves. High-resolution imagery of grooves will reveal subtle characteristics that will provide scientists with a lot more data to go off of, and could settle the debate entirely. The trailing face of Phobos has a region largely devoid of grooves, though it is possible that this is because it receives dust from Deimos that could bury grooves. This is my personal theory, and has not, to my knowledge, been acknowledged, but the rather high exchange rate means it is possible, depending on how and when grooves formed. Subsurface sounding may reveal these, or other hidden features!
![]() |
Credit: NASA and ESA |
![]() |
Credit: Brown University |
![]() |
Alignment of grooves with tidal stress. Source: Brown University |
That's it for now, thanks for reading another long post!
-Daniel
*DIFP
Thursday, March 16, 2017
PALADIN instrument orientation
What effect will our fixed-wheel design have on the nanorover's design?
A brief detail - our non-roving design is called DELPHI, for Deimos Exploration; Landing and PHobos Investigation. Our nanorover design, which will be detailed in this post, is called PALADIN, for Phobos Automotive Lander And Deimos INvestigation.
Most rovers that are equipped with an Alpha Proton X-ray Spectrometer have it on the end of an arm, so that it can be held against a specific target. MUSES-CN did not, but it was able to turn its body to press against a target. PALADIN, however, has a fixed AXS, held within the body at the proper distance from the surface. This is fine for measuring the composition of the surface regolith, but how will we measure the composition of ejecta blocks*, such as the Phobos Monolith, our primary target?
First, we have an optical spectrometer mounted on the front of the nanorover. By pointing the nanorover towards a boulder, we can take its spectra. An oversized momentum wheel allows the entire nanorover to be tilted up or down as required, pointing the spectrometer any desired target.
Second, on Eros, "ponds" were spotted around boulders (source). These ponds seem to be eroded boulder material, probably due primarily to the expansion and contraction of the boulder between day-night cycles. This seems to suggest that the upper layer of the regolith next to an ejecta block would closely match the composition of the block itself. Thus, by getting close to a block and taking the composition of the surface adjacent to it, the composition of the block can at least be inferred.
The NAC will point forward on the same axis as the optical spectrometer, and will be pointed in the same manner. LiDAR will, if possible, also point forwards, allowing distance measurements and mapping. The microscope will point directly downwards and will be located near the AXS. WAC angles have yet to be decided; one will point forward to provide context for the NAC, and other WACs may be included to provide multiple fields of view.
-Daniel
*DIFP
A brief detail - our non-roving design is called DELPHI, for Deimos Exploration; Landing and PHobos Investigation. Our nanorover design, which will be detailed in this post, is called PALADIN, for Phobos Automotive Lander And Deimos INvestigation.
Most rovers that are equipped with an Alpha Proton X-ray Spectrometer have it on the end of an arm, so that it can be held against a specific target. MUSES-CN did not, but it was able to turn its body to press against a target. PALADIN, however, has a fixed AXS, held within the body at the proper distance from the surface. This is fine for measuring the composition of the surface regolith, but how will we measure the composition of ejecta blocks*, such as the Phobos Monolith, our primary target?
First, we have an optical spectrometer mounted on the front of the nanorover. By pointing the nanorover towards a boulder, we can take its spectra. An oversized momentum wheel allows the entire nanorover to be tilted up or down as required, pointing the spectrometer any desired target.
Second, on Eros, "ponds" were spotted around boulders (source). These ponds seem to be eroded boulder material, probably due primarily to the expansion and contraction of the boulder between day-night cycles. This seems to suggest that the upper layer of the regolith next to an ejecta block would closely match the composition of the block itself. Thus, by getting close to a block and taking the composition of the surface adjacent to it, the composition of the block can at least be inferred.
![]() |
Credit: Icarus |
The NAC will point forward on the same axis as the optical spectrometer, and will be pointed in the same manner. LiDAR will, if possible, also point forwards, allowing distance measurements and mapping. The microscope will point directly downwards and will be located near the AXS. WAC angles have yet to be decided; one will point forward to provide context for the NAC, and other WACs may be included to provide multiple fields of view.
-Daniel
*DIFP
Monday, March 13, 2017
Focus On: Orbits
A quick post about the different kinds of orbits our mission uses!
QSO is used for mapping and reconnaissance from a safe, stable vantage point. This type of orbit allows for quick mapping of a moon and is stable over long periods, requiring very little stationkeeping. However, in order to remain stable, the craft must remain far enough away from the moon that the moon's gravity does not perturb its orbit. This means a craft in QSO of Phobos must remain about 30 km away. QSOs of Deimos may be slightly closer due to its lower mass.
Transitions between these orbits will be detailed when I actually understand them.
QSO
Because of the moons' low mass and proximity to Mars, actually orbiting them is nearly impossible. However, it is possible to enter a "Quasi-Synchronous Orbit" (QSO) where the craft is actually orbiting Mars in a slightly eccentric orbit in such a way that it circles the moon.
![]() |
Credit: Instituto Superior Técnico |
![]() |
Credit: Instituto Superior Técnico |
QSO is used for mapping and reconnaissance from a safe, stable vantage point. This type of orbit allows for quick mapping of a moon and is stable over long periods, requiring very little stationkeeping. However, in order to remain stable, the craft must remain far enough away from the moon that the moon's gravity does not perturb its orbit. This means a craft in QSO of Phobos must remain about 30 km away. QSOs of Deimos may be slightly closer due to its lower mass.
LPO
Because of the moons' low mass and their proximity to Mars, Phobos's Lagrange point, where the gravity of Mars and Phobos cancel each other out (in this context), is 2-3 km from Phobos's surface. This allows for proximity investigation of Phobos's surface, over a hundred times closer than Earth-based CubeSats ever get to their targets, and over ten times closer than the closest Phobos flyby to date.
A spacecraft at a Lagrange point is like a marble on top of a hill - while it takes relatively little energy to keep it there, without correction it will slowly drift off. A craft in an LPO can take very accurate measurements and extremely high-resolution images of Phobos, but the stationkeeping required to keep it there is rather difficult, especially without the use of ground-based radio telescopes. Most likely the spacecraft will spend a relatively short time in LPO before safely flying out or landing. Thankfully, an LPO can stretch across a large region around the Lagrange point, and can pass over many objects of interest, such as the Phobos Monolith, Stickney Crater, and a number of grooves *.
Artificial LPO
Because of the moons' low mass and their proximity to Mars, Phobos's Lagrange point, where the gravity of Mars and Phobos cancel each other out (in this context), is 2-3 km from Phobos's surface. This allows for proximity investigation of Phobos's surface, over a hundred times closer than Earth-based CubeSats ever get to their targets, and over ten times closer than the closest Phobos flyby to date.
A spacecraft at a Lagrange point is like a marble on top of a hill - while it takes relatively little energy to keep it there, without correction it will slowly drift off. A craft in an LPO can take very accurate measurements and extremely high-resolution images of Phobos, but the stationkeeping required to keep it there is rather difficult, especially without the use of ground-based radio telescopes. Most likely the spacecraft will spend a relatively short time in LPO before safely flying out or landing. Thankfully, an LPO can stretch across a large region around the Lagrange point, and can pass over many objects of interest, such as the Phobos Monolith, Stickney Crater, and a number of grooves *.
![]() |
Credit: Mattia Zamaro |
Artificial LPO
By applying constant thrust in one direction, the stability of an LPO can be significantly increased and orbits can be moved closer to the surface. While propellant-intensive, such an orbit can be used to facilitate a very low-velocity landing*.
Transitions between these orbits will be detailed when I actually understand them.
For more details on orbits of the Martian moons, read Mattia Zamaro's thesis.
-Daniel
*DIFP
Friday, March 10, 2017
Focus On: Instruments
What do we want to do once we get to the moons? What instruments will we be carrying?
Orbital instruments
The most important instrument is a Narrow-Angle Camera! This camera gives us high-resolution imagery of both moons. Current CubeSat imagers are more than powerful enough, as we can get far closer to the moons than CubeSats can ever get to the Earth's surface. Our first choice right now is GOMspace's NanoCam C1U (datasheet).
With a 70 mm lens, it masses about 277 grams and takes up most of 1U ( = 10 x 10 x 10 cm), although the space next to the lens may be used for flat components such as batteries. At a range of 30 km, which is our mapping distance for both moons*, we get a resolution of about 132 cm/pixel, four times better than the current best images. At a distance of 2.5 km, which is our proximity imaging distance*, we get a resolution of about 11 cm/pixel, which is amazing. However, this is a narrow-angle camera, and so has a limited field of view, with a swath of about 2 km at mapping, and 200 m at proximity.
Our second choice for a NAC is the SCS Gecko (brochure), which has about half the resolution, significantly shorter, and about twice as heavy.
A smaller, wide-angle camera (WAC) is also important to give context to high-resolution imagery and to allow navigation via craters. We may include multiple WACs pointing in different directions. There are a number of options we are considering, and these cameras are rather common among CubeSats. The model we are currently planning to use is the Crystalspace CAM1U (datasheet).
A laser altimeter is necessary for stationkeeping and landing, but ideally we would want a LiDAR which would let us map Phobos (We may be keeping our distance from Deimos, so likely wouldn't get close enough for LiDAR to work*). Since most CubeSats remain in LEO, where LiDAR isn't especially easy or useful, not a lot of work has been done, except for development of short-range LiDARs for rendezvous and formation flying. SPEC was working on a 0.5 U LiDAR (proposal) in 2014 that had a range of 8 km, a 30 degree field of view, and an integrated camera. Georgia Tech is currently working on a LiDAR CubeSat that is accurate to a few centimeters over ranges of tens of kilometers.
Although both moons' spectra has been taken in the past, a spectrometer working together with the NAC or with imaging capabilities would be able to find the spectra of specific targets and map the different regions of the moons. We are currently looking into a hyperspectral imager developed in Finland (presentation), which would take pictures at different wavelengths, returning a "data cube" with the spectra of each pixel.
However, this imager is still in early stages of development, and may require significant modification to work for our mission, so it may not be feasible. We also plan to include the Argus 1000 IR spectrometer (datasheet) which takes a much higher-resolution spectra of a single point. This smaller instrument is 45 x 50 x 80 mm and masses 215 grams. The spectrometer has a spectral range of 900 nm to 1700 nm, with an extended version reaching 2400 nm. It will point along the axis of the NAC, so we can see exactly what the spectrometer measures.
Landed instruments
If we opt for a simplified mission plan*, most of the following instruments will not be included, although some may be, as a landing would still be attempted towards the end of the mission. In this case, it is possible that orbital instruments will be expanded, depending on the volume available.
One reason so little is known of Phobos and Deimos's composition is that they both have very ambiguous spectra. An Alpha-X-ray Spectrometer, however, is able to directly measure the elemental composition of all elements (except hydrogen and helium). An AXS built for the MUSES-CN mission fits within 65 cubic centimeters, masses only 95 grams, and takes 30 minutes to three hours to take a measurement. This instrument was built nearly 20 years ago, and Dr. Economou, the primary investigator for the AXS, is working on an updated version of the AXS with significantly higher accuracy, smaller size, and shorter accumulation time.
A lander is uniquely capable of taking extremely high-resolution images of regolith. In a pinch, a WAC with special optics will do, but ideally we would wish to include a microscope. We initially planned to have a MicrOmega imaging spectroscope (details), which would allow the spectra of each dust particle to be measured, but despite its obvious value, MicrOmega is slightly too large to feasibly include without redesigning it and removing other instruments.
The microscope built for the failed Beagle 2 mission is similar in resolution and significantly smaller, although not able to take spectra. It masses about 245 grams and measures 111 x 52 x 22 mm and takes images about 4 x 4 mm, with a resolution of 4 microns per pixel. This would have to be reduced slightly in size to be shorter than 100 mm, and would need to have a longer focal length to work with a rover*. Some loss in performance is likely to be expected, since minimizing cost and size is more important than reaching some specific resolution.
Some less-likely instruments include a muograph (paper) and a radio sounding instrument (paper) which would allow mapping Phobos's interior to a depth of a few hundred meters; dust adhesion/electrical rejection and regolith compression and cohesion experiments to investigate regolith properties; a thermal infrared imager, thermal radiometer, or temperature probe to investigate heat flow; or dedicated gravitometers or magnetometers to measure the gravitational and magnetic environment.
Thermometers, magnetometers, and accelerometers on the flight computer can give rough measurements without any dedicated instruments.
Sorry for another lengthy post, I'm trying to get all the mission details into the public domain. The next few will be shorter, I promise! Coming up next- Considerations for a fixed-wheel rover.
-Daniel
*Detailed In Future Post (DIFP)
Orbital instruments
The most important instrument is a Narrow-Angle Camera! This camera gives us high-resolution imagery of both moons. Current CubeSat imagers are more than powerful enough, as we can get far closer to the moons than CubeSats can ever get to the Earth's surface. Our first choice right now is GOMspace's NanoCam C1U (datasheet).
![]() |
Credit: GOMspace
|
With a 70 mm lens, it masses about 277 grams and takes up most of 1U ( = 10 x 10 x 10 cm), although the space next to the lens may be used for flat components such as batteries. At a range of 30 km, which is our mapping distance for both moons*, we get a resolution of about 132 cm/pixel, four times better than the current best images. At a distance of 2.5 km, which is our proximity imaging distance*, we get a resolution of about 11 cm/pixel, which is amazing. However, this is a narrow-angle camera, and so has a limited field of view, with a swath of about 2 km at mapping, and 200 m at proximity.
Our second choice for a NAC is the SCS Gecko (brochure), which has about half the resolution, significantly shorter, and about twice as heavy.
A smaller, wide-angle camera (WAC) is also important to give context to high-resolution imagery and to allow navigation via craters. We may include multiple WACs pointing in different directions. There are a number of options we are considering, and these cameras are rather common among CubeSats. The model we are currently planning to use is the Crystalspace CAM1U (datasheet).
A laser altimeter is necessary for stationkeeping and landing, but ideally we would want a LiDAR which would let us map Phobos (We may be keeping our distance from Deimos, so likely wouldn't get close enough for LiDAR to work*). Since most CubeSats remain in LEO, where LiDAR isn't especially easy or useful, not a lot of work has been done, except for development of short-range LiDARs for rendezvous and formation flying. SPEC was working on a 0.5 U LiDAR (proposal) in 2014 that had a range of 8 km, a 30 degree field of view, and an integrated camera. Georgia Tech is currently working on a LiDAR CubeSat that is accurate to a few centimeters over ranges of tens of kilometers.
Although both moons' spectra has been taken in the past, a spectrometer working together with the NAC or with imaging capabilities would be able to find the spectra of specific targets and map the different regions of the moons. We are currently looking into a hyperspectral imager developed in Finland (presentation), which would take pictures at different wavelengths, returning a "data cube" with the spectra of each pixel.
![]() |
Credit: VTT |
However, this imager is still in early stages of development, and may require significant modification to work for our mission, so it may not be feasible. We also plan to include the Argus 1000 IR spectrometer (datasheet) which takes a much higher-resolution spectra of a single point. This smaller instrument is 45 x 50 x 80 mm and masses 215 grams. The spectrometer has a spectral range of 900 nm to 1700 nm, with an extended version reaching 2400 nm. It will point along the axis of the NAC, so we can see exactly what the spectrometer measures.
Credit: Thoth Technology |
Landed instruments
If we opt for a simplified mission plan*, most of the following instruments will not be included, although some may be, as a landing would still be attempted towards the end of the mission. In this case, it is possible that orbital instruments will be expanded, depending on the volume available.
One reason so little is known of Phobos and Deimos's composition is that they both have very ambiguous spectra. An Alpha-X-ray Spectrometer, however, is able to directly measure the elemental composition of all elements (except hydrogen and helium). An AXS built for the MUSES-CN mission fits within 65 cubic centimeters, masses only 95 grams, and takes 30 minutes to three hours to take a measurement. This instrument was built nearly 20 years ago, and Dr. Economou, the primary investigator for the AXS, is working on an updated version of the AXS with significantly higher accuracy, smaller size, and shorter accumulation time.
![]() |
Credit: University of Chicago |
A lander is uniquely capable of taking extremely high-resolution images of regolith. In a pinch, a WAC with special optics will do, but ideally we would wish to include a microscope. We initially planned to have a MicrOmega imaging spectroscope (details), which would allow the spectra of each dust particle to be measured, but despite its obvious value, MicrOmega is slightly too large to feasibly include without redesigning it and removing other instruments.
![]() |
Credit: CNES |
The microscope built for the failed Beagle 2 mission is similar in resolution and significantly smaller, although not able to take spectra. It masses about 245 grams and measures 111 x 52 x 22 mm and takes images about 4 x 4 mm, with a resolution of 4 microns per pixel. This would have to be reduced slightly in size to be shorter than 100 mm, and would need to have a longer focal length to work with a rover*. Some loss in performance is likely to be expected, since minimizing cost and size is more important than reaching some specific resolution.
![]() |
Credit: Beagle 2 |
Some less-likely instruments include a muograph (paper) and a radio sounding instrument (paper) which would allow mapping Phobos's interior to a depth of a few hundred meters; dust adhesion/electrical rejection and regolith compression and cohesion experiments to investigate regolith properties; a thermal infrared imager, thermal radiometer, or temperature probe to investigate heat flow; or dedicated gravitometers or magnetometers to measure the gravitational and magnetic environment.
Thermometers, magnetometers, and accelerometers on the flight computer can give rough measurements without any dedicated instruments.
Sorry for another lengthy post, I'm trying to get all the mission details into the public domain. The next few will be shorter, I promise! Coming up next- Considerations for a fixed-wheel rover.
-Daniel
*Detailed In Future Post (DIFP)
Thursday, March 9, 2017
Focus on: Mobility
First things first, why do we want a nanorover? MarMoSet is already really ambitious, so why make things much more complicated?
The fact is, if we go to all the trouble of sending a probe to Phobos, we absolutely want to land. An orbiter is still invaluable, but landing, while not mission-critical, would vastly increase the mission's science return. Ground-truthing can ensure that data collected from orbit is accurate. Many types of data, such as microscopy (which is important to understanding Phobos's nature and environment), non-optical spectrometry (which can directly measure composition), and regolith properties (critical to know before a crewed mission), can only be taken at the surface. And regardless of whether the landing succeeds or fails, the attempt itself will be a source of data for later, more-capable (and expensive!) missions.
Even our non-lander concept includes a landing at the end of the mission, but this is a why-not, planetary-protection, see-what-we-can-get landing. Designing the probe to reliably survive a landing without unduly compromising the rest of the mission is a different matter. Wheels actually make a landing easier, since landings on Phobos can be at a very shallow angle, to be detailed in a future post (DIFP). Landings are likely to be on the order of a few meters per second; wheels allow us to choose a trajectory that minimizes vertical speed, and the wheels, spun up to match the probe's horizontal speed, can decelerate the probe over time, minimizing stresses. In a worst-case scenario, wheels act as a crumple zone, protecting the rest of the probe and allowing some data to be returned from the surface.
In addition to helping the probe survive landing, wheels would of course allow the probe to rove over Phobos's surface. While many approaches more suited to milligravity have been proposed, such as hoppers, cilia, and legs, these approaches all require the rover to be designed around them. Wheels minimally impact the design of the probe, and can draw from the extensive development of nanorovers for both space and terrestrial applications.
Roving significantly increases science return (details). Not only does it allow fine positioning and investigation of multiple sites of interest, it permits us to investigate the interaction between different regions, which can reveal a great deal about the nature of Phobos. Specifically, looking at the interaction between Phobos's color units (DIFP) can help determine its interior composition.
The mission most similar to our plan is the MUSES-CN nanorover, developed by JPL for the Hayabusa mission. While it was cancelled due to cost and weight overruns, there was plenty of development for us to learn from. Mission details can be found here.
MUSES-CN was designed to rove around on the asteroid Itokawa. Its expected top speed was about 1 mm/s, but Phobos is a much nicer environment for a nanorover than Itokawa. With a surface gravity 50 times higher, nanorovers have more friction to push with, and can go much faster without worrying about escaping the body. Phobos's surface is also much smoother than Itokawa's, due to a thick layer of dust (DIFP).
The fact is, if we go to all the trouble of sending a probe to Phobos, we absolutely want to land. An orbiter is still invaluable, but landing, while not mission-critical, would vastly increase the mission's science return. Ground-truthing can ensure that data collected from orbit is accurate. Many types of data, such as microscopy (which is important to understanding Phobos's nature and environment), non-optical spectrometry (which can directly measure composition), and regolith properties (critical to know before a crewed mission), can only be taken at the surface. And regardless of whether the landing succeeds or fails, the attempt itself will be a source of data for later, more-capable (and expensive!) missions.
Even our non-lander concept includes a landing at the end of the mission, but this is a why-not, planetary-protection, see-what-we-can-get landing. Designing the probe to reliably survive a landing without unduly compromising the rest of the mission is a different matter. Wheels actually make a landing easier, since landings on Phobos can be at a very shallow angle, to be detailed in a future post (DIFP). Landings are likely to be on the order of a few meters per second; wheels allow us to choose a trajectory that minimizes vertical speed, and the wheels, spun up to match the probe's horizontal speed, can decelerate the probe over time, minimizing stresses. In a worst-case scenario, wheels act as a crumple zone, protecting the rest of the probe and allowing some data to be returned from the surface.
In addition to helping the probe survive landing, wheels would of course allow the probe to rove over Phobos's surface. While many approaches more suited to milligravity have been proposed, such as hoppers, cilia, and legs, these approaches all require the rover to be designed around them. Wheels minimally impact the design of the probe, and can draw from the extensive development of nanorovers for both space and terrestrial applications.
Roving significantly increases science return (details). Not only does it allow fine positioning and investigation of multiple sites of interest, it permits us to investigate the interaction between different regions, which can reveal a great deal about the nature of Phobos. Specifically, looking at the interaction between Phobos's color units (DIFP) can help determine its interior composition.
The mission most similar to our plan is the MUSES-CN nanorover, developed by JPL for the Hayabusa mission. While it was cancelled due to cost and weight overruns, there was plenty of development for us to learn from. Mission details can be found here.
![]() |
Credit: JPL |
![]() |
Phobos surface. MOC image SP2-55103, credit NASA. |
![]() |
Itokawa - credit JAXA, ISAS |
So how fast can we go on Phobos? Acceleration is limited by surface gravity and surface cohesion. We haven't crunched any numbers yet, but the surface gravity near our landing site is about 4 mm/s^2, so acceleration would be some fraction of that. Top speed, however, is much less limited. Obviously, top speed should be kept significantly under escape velocity, to avoid accidentally escaping the body. For MUSES-CN, Itokawa's low escape velocity of 15 cm/s meant that speeds on the order of centimeters per second were risky , while on Phobos traverses of tens of centimeters per second could be possible.
The radius of curvature of the surface must also be taken into account. A rover can go pretty fast inside a crater, but has to slow down on the rim to avoid flying off. However, on the planetary scale, this only limits the rover to about a meter per second, well over expected speeds. Speeds will be more limited at the rim of small craters and grooves, which will have to be taken into consideration. Since impact speeds would approximately equal takeoff speeds, though, ridges could be used to jump large distances quickly, although the risk inherent here, even with reaction wheel control, means that such a gambit would only be attempted late in the mission, after all primary objectives have been reached.
![]() |
Credit: D.J. Scheeres |
The primary reason to keep speed down is that the faster the rover goes, the longer it will lose contact with the surface after hitting a bump. This is even less of an issue for us, since our nanorover would have reaction wheels and so would have some control without surface contact. The speed of our nanorover will be constrained primarily by the available motors. We believe speeds on the order of ten centimeters per second to be reasonable, but even speeds of a centimeter per second allow long traverses.
We initially intended to use MUSES-CN's wheels, as they were developed almost to the point of flight-readiness. However, the wheels need to be stowed within the frame to be deployed. Having the wheels fold out was complex and took up a lot of internal volume. We decided against articulated wheels after learning that MUSES-CN's struts were not meant to move to maintain contact with the surface, but only to allow the body to be rotated. The effect of fixed wheels will be discussed in an upcoming post.
We decided to take a new approach to wheels, with the goal of minimizing internal volume. We currently plan to use wheels similar to those used by the LAMAlice nanorover (details), with the primary difference being that our wheels would be slightly larger, at approximately 6 cm in diameter. Other adjustments, such as changing the number or thickness of blades, may be made as we get a better understanding of their effect on performance. The wheels would be stowed inside the nanorover as shown at the right. Our current approach to deployment is as follows:
We initially intended to use MUSES-CN's wheels, as they were developed almost to the point of flight-readiness. However, the wheels need to be stowed within the frame to be deployed. Having the wheels fold out was complex and took up a lot of internal volume. We decided against articulated wheels after learning that MUSES-CN's struts were not meant to move to maintain contact with the surface, but only to allow the body to be rotated. The effect of fixed wheels will be discussed in an upcoming post.
We decided to take a new approach to wheels, with the goal of minimizing internal volume. We currently plan to use wheels similar to those used by the LAMAlice nanorover (details), with the primary difference being that our wheels would be slightly larger, at approximately 6 cm in diameter. Other adjustments, such as changing the number or thickness of blades, may be made as we get a better understanding of their effect on performance. The wheels would be stowed inside the nanorover as shown at the right. Our current approach to deployment is as follows:
![]() |
Credit: EPFL |
The drive shaft is a rod with helical flutes, like a drill bit. While within the nanorover's frame, the wheel is prevented from turning by a rod between the spokes, so as the drive shaft is turned, the wheel is pushed outside the nanorover's frame, and the blades spring open. A cap at the end of the shaft prevents the wheel from moving further out, and the open blades prevent it from moving in. Now, when the shaft is turned, the wheel turns with it.
The main difficulty with this design is that the shaft cannot extend outside the frame. Currently we plan to have the wheel attached to a helical spline which remains attached to the drive shaft. Unfortunately, this does somewhat increase the required internal volume, as the shaft must be at least twice as long as the wheel is wide.
Each wheel will be powered by its own motor. Due to the low gravity of Phobos, it's very important for the motor to rotate in very small increments. MUSES-CN's motors could be rotated by a mere 0.044 degrees, moving forward only 23 microns per step! Consistency and control is much more important than power or speed. While we haven't chosen a motor yet, it will likely be very similar to those of MUSES-CN or Sojourner.
It's very difficult to predict how long the probe will survive on the surface. It could fail almost immediately, or remain functional for months. The main life-limiting factor is expected to be thermal cycling, as the nanorover would go through large temperature swings every seven hours. MUSES-CN, with minimal thermal control, was expected to survive about 30 days; our nanorover will likely have better thermal control, but will not be designed as a nanorover, so we really don't know what to expect. We will probably have a better lifetime estimate closer to the mission. This means it's very important to prioritize targets, to make sure the most important targets are investigated first. The landing site will be selected based on ease of landing, but we plan to land close to the Phobos Monolith, which can be seen in the image of Phobos's surface. After that, we plan to traverse the grooves, move around Stickney Crater visiting boulders and craters, and towards the end of the mission investigate landslide features and other riskier targets. All of this will, of course, be DIFP. The mission is unlikely to extend past October 2021, as at this point the Sun will come between Earth and Mars, and communication with Mars will be lost. If most objectives can be met before then, it's unlikely that we will attempt to reestablish communication.
Whew! This was quite a lengthy post. Most posts will be much more readable than this was, but I've been asked multiple times about the wheels, and I wanted to get all the details out there into the public. Thank you for reading!
-Daniel Zsenits
The main difficulty with this design is that the shaft cannot extend outside the frame. Currently we plan to have the wheel attached to a helical spline which remains attached to the drive shaft. Unfortunately, this does somewhat increase the required internal volume, as the shaft must be at least twice as long as the wheel is wide.
Each wheel will be powered by its own motor. Due to the low gravity of Phobos, it's very important for the motor to rotate in very small increments. MUSES-CN's motors could be rotated by a mere 0.044 degrees, moving forward only 23 microns per step! Consistency and control is much more important than power or speed. While we haven't chosen a motor yet, it will likely be very similar to those of MUSES-CN or Sojourner.
It's very difficult to predict how long the probe will survive on the surface. It could fail almost immediately, or remain functional for months. The main life-limiting factor is expected to be thermal cycling, as the nanorover would go through large temperature swings every seven hours. MUSES-CN, with minimal thermal control, was expected to survive about 30 days; our nanorover will likely have better thermal control, but will not be designed as a nanorover, so we really don't know what to expect. We will probably have a better lifetime estimate closer to the mission. This means it's very important to prioritize targets, to make sure the most important targets are investigated first. The landing site will be selected based on ease of landing, but we plan to land close to the Phobos Monolith, which can be seen in the image of Phobos's surface. After that, we plan to traverse the grooves, move around Stickney Crater visiting boulders and craters, and towards the end of the mission investigate landslide features and other riskier targets. All of this will, of course, be DIFP. The mission is unlikely to extend past October 2021, as at this point the Sun will come between Earth and Mars, and communication with Mars will be lost. If most objectives can be met before then, it's unlikely that we will attempt to reestablish communication.
Whew! This was quite a lengthy post. Most posts will be much more readable than this was, but I've been asked multiple times about the wheels, and I wanted to get all the details out there into the public. Thank you for reading!
-Daniel Zsenits
Thursday, March 2, 2017
More mission details!
I won't be able to post any more mission details until Sunday due to college application and scholarship stuff, so if you're interested, please check out the presentation I gave at Oxford last year!
Thank you all for your interest in MarMoSet!
-Daniel
Thank you all for your interest in MarMoSet!
-Daniel
Tuesday, February 28, 2017
Focus On: Propulsion
The two most important metrics when considering propulsion for this mission are exhaust velocity and thrust per watt. A higher exhaust velocity reduces the propellant needed for a mission, while higher thrust per watt increases performance or reduces the required electric power.
Most CubeSat propulsion available today uses pressurized gas or monopropellant. While most of these require nearly no power to run, they have very low exhaust velocities. On the other end of the spectrum, most electric thrusters have excellent exhaust velocities, but are heavy, require a great deal of power, and provide very little thrust.
Our research into this mission first became serious when we discovered the Cubesat Ambipolar Thruster (CAT), which is designed to enable interplanetary CubeSat missions. Permanent magnets form a magnetic nozzle, and an RF antenna creates plasma.
CAT uses iodine as propellant, which has performance very close to that of xenon, and is cheaper and can be stored solid. Solid storage means that propellant tanks can be much smaller and do not have to hold pressure.
CAT provides about 100 mN/kW, as opposed to 10 from comparable ion thrusters, at about 1,000sec Isp, compared to 200 from monopropellant thrusters. However, while efficient enough to allow a CubeSat to get to Mars by itself(!), large solar arrays would need to be custom-made to provide enough power for the thruster to give enough thrust for practical operations at Mars, where solar intensity is 57%-71% lower than at Earth.
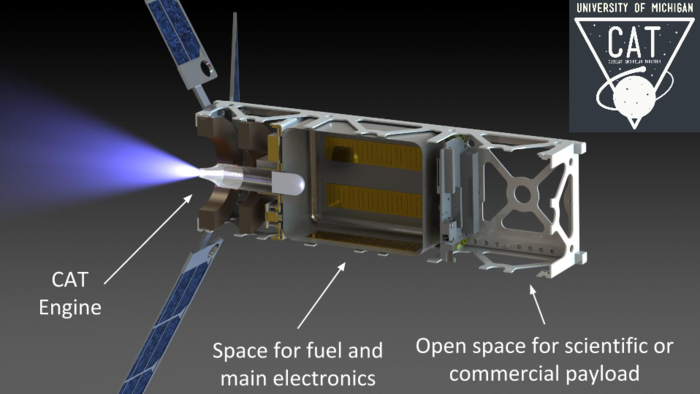
Credit: University of Michigan
Last November, we came across the ConstantQ hybrid electrostatic thruster, which is smaller, more efficient, and higher-performance than CAT for our purposes (CAT is still an excellent option for other applications!). The standard Model H system is comprised of four slightly angled thruster heads. The angle allows differential thrusting to provide torque, which is useful for desaturating reaction wheels (allowing flywheels to gradually slow down to nominal speeds without turning the spacecraft)

Credit: Fluid and Reason, LLC
The thruster generates plasma in pulses, about 2400 times per second. While listed as providing 1.25 mN of thrust per head, at 760sec Isp, tests have shown that it can provide 1.6 mN of thrust at 1,100sec Isp, which gives us a whopping 290.9 mN/kW at a better exhaust velocity than the CAT.
This increased thrust also means that 4 thruster heads give us enough acceleration, so we can use the off-the-shelf model of the thruster. The Model H masses 500 grams without propellant. Like the CAT, the ConstantQ uses iodine propellant which is stored solid, at a density of about 4 g/cm^3.
We expect the propulsion system to fit within 10 cm x 10 cm x 12 cm and carry slightly over two kilograms of propellant. Having a quarter of the initial spacecraft mass be propellant provides us with 3 km/s of delta-V, which we estimate is more than enough to complete our mission after Mars injection.
A solo mission to Mars from Geostationary Earth Orbit is feasible, but would require 50%-60% of the spacecraft's mass to be propellant, and would pose challenges that will be detailed in a future post.
Upcoming post: mission profiles!
Most CubeSat propulsion available today uses pressurized gas or monopropellant. While most of these require nearly no power to run, they have very low exhaust velocities. On the other end of the spectrum, most electric thrusters have excellent exhaust velocities, but are heavy, require a great deal of power, and provide very little thrust.
Our research into this mission first became serious when we discovered the Cubesat Ambipolar Thruster (CAT), which is designed to enable interplanetary CubeSat missions. Permanent magnets form a magnetic nozzle, and an RF antenna creates plasma.
CAT uses iodine as propellant, which has performance very close to that of xenon, and is cheaper and can be stored solid. Solid storage means that propellant tanks can be much smaller and do not have to hold pressure.
CAT provides about 100 mN/kW, as opposed to 10 from comparable ion thrusters, at about 1,000sec Isp, compared to 200 from monopropellant thrusters. However, while efficient enough to allow a CubeSat to get to Mars by itself(!), large solar arrays would need to be custom-made to provide enough power for the thruster to give enough thrust for practical operations at Mars, where solar intensity is 57%-71% lower than at Earth.
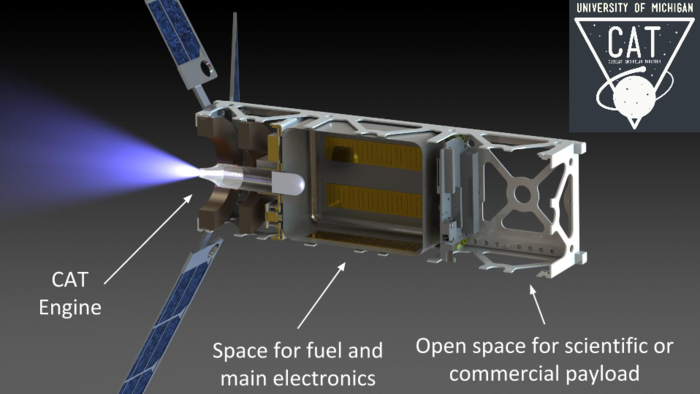
Credit: University of Michigan
Last November, we came across the ConstantQ hybrid electrostatic thruster, which is smaller, more efficient, and higher-performance than CAT for our purposes (CAT is still an excellent option for other applications!). The standard Model H system is comprised of four slightly angled thruster heads. The angle allows differential thrusting to provide torque, which is useful for desaturating reaction wheels (allowing flywheels to gradually slow down to nominal speeds without turning the spacecraft)
Credit: Fluid and Reason, LLC
The thruster generates plasma in pulses, about 2400 times per second. While listed as providing 1.25 mN of thrust per head, at 760sec Isp, tests have shown that it can provide 1.6 mN of thrust at 1,100sec Isp, which gives us a whopping 290.9 mN/kW at a better exhaust velocity than the CAT.
"Gas flow rate and the timing of these pulses drive the performance. When the flow rate goes too low, no plasma is generated. When too high, cold gas thrust increases but no plasma is formed. It's a very large flow range though. Likewise, when the spark frequency is too low, too little plasma is formed to combat the neutral gas and no plasma gets accelerated. When the spark frequency is too high, shock waves in the gas stop propellant flow and thrust stops. We have a variety of patent pending feedback mechanisms that let us control the operating point in real time." - Wes Faler
This increased thrust also means that 4 thruster heads give us enough acceleration, so we can use the off-the-shelf model of the thruster. The Model H masses 500 grams without propellant. Like the CAT, the ConstantQ uses iodine propellant which is stored solid, at a density of about 4 g/cm^3.
We expect the propulsion system to fit within 10 cm x 10 cm x 12 cm and carry slightly over two kilograms of propellant. Having a quarter of the initial spacecraft mass be propellant provides us with 3 km/s of delta-V, which we estimate is more than enough to complete our mission after Mars injection.
A solo mission to Mars from Geostationary Earth Orbit is feasible, but would require 50%-60% of the spacecraft's mass to be propellant, and would pose challenges that will be detailed in a future post.
Upcoming post: mission profiles!
Monday, February 27, 2017
The Story Thus Far
MarMoSet was born just over a year ago. In December of 2015, while talking to a friend about the Google Lunar XPrize, I remembered that it's theoretically easier to land on Mars's moons than our own. For fun, we began looking into what it would take to send a small mission to the Martian moons.
Once we discovered other ambitious CubeSat missions and the technology that enabled them, we realized that such a mission was actually possible. This led us to start taking the mission seriously and look into the details of such a mission.
While researching work being done on similar missions, I came across iCubeSat, a workshop on interplanetary CubeSat missions, science, and technology. I submitted an abstract and ended up giving a presentation at Oxford in late May. You can find that presentation here.
In July we attended The Third International Conference on the Exploration of Phobos and Deimos. All the presentations from there are available at the site. Most importantly, we learned that SpaceX's Red Dragon mission has up to 500 kg available for secondary payloads, and that JAXA is planning a Phobos sample return to launch in 2022. We also met with Deep Space Industries to discuss their asteroid prospector missions.In November, after two months of trying to get in contact with SpaceX, I got an answer from an engineer leading the Red Dragon program confirming that our mission could piggyback to Mars on the Red Dragon.
We also discovered new propulsion systems and research that enabled us to start looking more in-depth at our mission plan.
We're now working on contacting researchers and on creating a detailed reference mission, as well as making the work we're doing more available to the public through this blog.
Coming soon, posts detailing:
Mission rationale! Why do we even want to go to Phobos?
Spacecraft systems! First on the list: propulsion!
Mission profile! What even IS MarMoSet?
-Daniel
Coming soon, posts detailing:
Mission rationale! Why do we even want to go to Phobos?
Spacecraft systems! First on the list: propulsion!
Mission profile! What even IS MarMoSet?
-Daniel
Subscribe to:
Posts (Atom)